How Does Gene Therapy Work?
Gene therapy is an exquisite emerging technology that deposits new DNA into faulty body cells to restore their function.
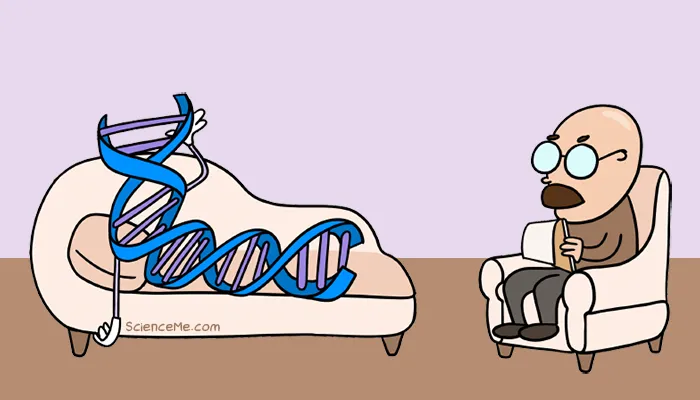
Treating disease at the fundamental level of DNA is sophisticated, yet it carries a host of knowns and unknowns. How do you transfer entire intact genes into cells? How do they become a permanent part of your body? And what happens when gene therapy goes wrong?
Before we look at how gene therapy works, you may want to know how DNA works. Go ahead and find out. I'll wait here.
What is Gene Therapy?
When you first heard of gene therapy, did you imagine every cell in your body getting a DNA upgrade? Because I did. But this isn't a sci-fi movie; we're not creating mutants (in fact, it's the opposite), no-one's going to gain any superpowers, and there shall be no dramatic changes to your body plan.
Gene therapy targets a single faulty cell type. It doesn't change the DNA in all your body cells—that would not only be much more difficult but also highly redundant.
This is called somatic gene therapy. It contrasts with germline gene therapy, which targets sperm and egg cells to eliminate errors from the gene pool. Germline changes are currently illegal due to the many potential dangers.
Similar to genetic vaccines, gene therapy uses vectors, or carriers, to transport healthy DNA into patient cells. Viruses make good natural vectors because they evolved to inject their genes into cells. Current research also targets synthetic vectors like lipid nanoparticles to deliver the DNA payload.
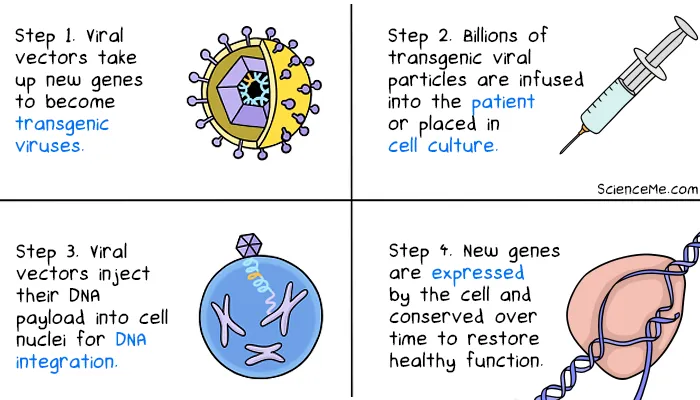
How gene therapy works: (1) vector creation; (2) gene delivery; (3) integration; (4) expression and conservation.
The first gene therapy was approved by the FDA in 2017 to treat retinal dystrophy, an eye disorder that causes progressive blindness. Since then, more than 20 cell and gene therapies have made it into general use.
The gene therapies under research today generally fall into two categories:
- Gene replacement adds either protein-coding genes to treat loss-of-function diseases like cystic fibrosis, or suicide genes that program apoptosis (cell death) to destroy cancerous tumours.
- Gene silencing blocks the action of faulty genes using RNA interference, either as a cancer therapy or to overcome drug resistance. Macromolecules called siRNA (small interfering RNA) bind to mRNA in cells and block protein synthesis.
Initially, advances in clinical research hope to treat rare but devastating monogenic (single gene) disorders, as well as incurable cancers. Eventually, this versatile technology will override many traditional drug treatments, offering permanent cures to life-long or life-ending diseases.
While each type of therapy is highly customised, let's consider the process of gene replacement therapy to treat a loss-of-function disease.
Step 1. Vector Creation
First we need a vector. This is any vehicle, like a virus or a nanoparticle, that can transport the genetic payload into cells.
Viral vectors exploit the natural drive for viruses to invade cells, deposit their genes for replication, and even integrate with the host DNA. In preparation for gene therapy, viral replication genes are edited out, which creates space in the viral genome to add therapeutic human genes.
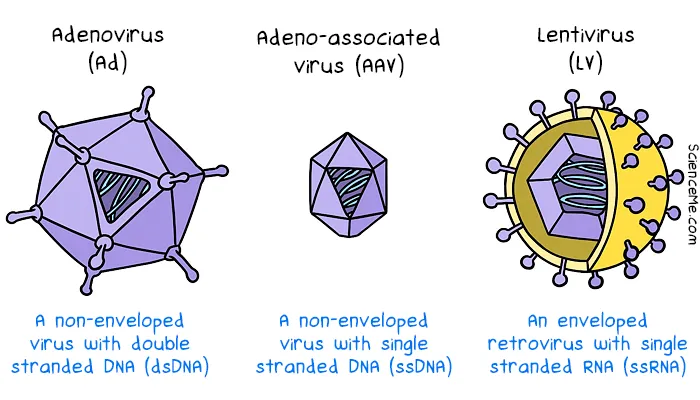
The most common viral vectors in gene therapy include adenoviruses (Ad), adeno-associated viruses (AAV), and lentiviruses (LV).
However, each family of viruses comes with their own benefits and risks. Choosing the best viral vector and dosage is a balancing act that determines the rate of DNA delivery, immune response, and integration errors.
Non-viral vectors include synthetic nanoparticles which can be designed from scratch to deliver DNA. While non-viral vectors reduce the risk of an overactive immune response, the tend to have lower rates of gene delivery. Nonetheless, synthetic vectors laid the groundwork for using lipid nanoparticle (LNP) vectors in mRNA vaccines.
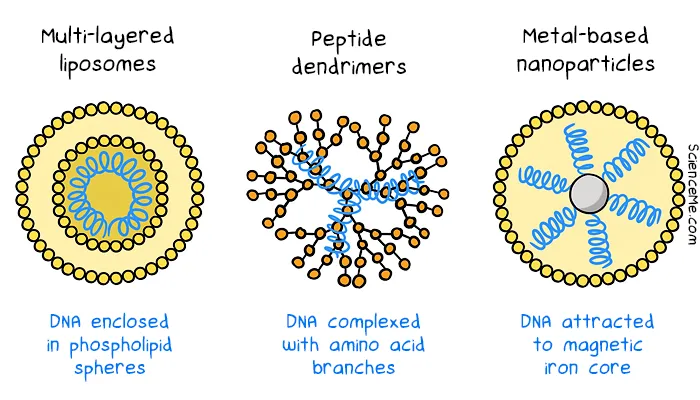
Non-viral vectors include liposomes, dendrimers, and metal-based nanoparticles.
Step 2. Gene Delivery
Now the vector is introduced to the body or a cell culture in the lab to deliver the genetic cargo. This is called transduction in viral vectors and transfection in non-viral vectors.
When a vector meets a cell, it must successfully gain entry at the cell membrane and deposit the DNA payload, all without triggering a major intracellular immune response that destroys the cell.
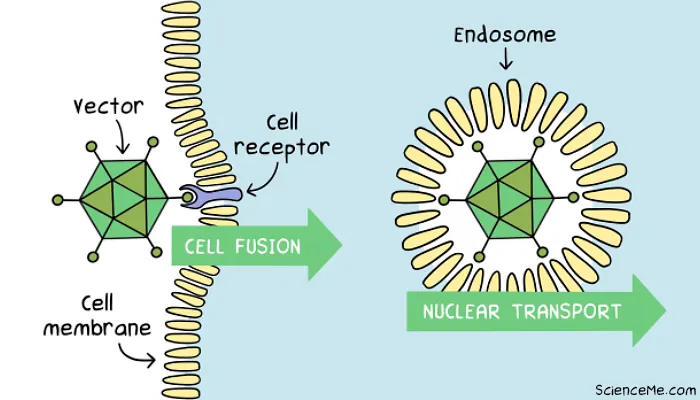
Transduction sees the viral vector introduce foreign DNA into cells, first by binding with a cell membrane receptor, then being absorbed by a lipid bubble called an endosome which carries it to the nucleus.
- In vivo gene therapy sees vectors infused into the bloodstream so they can target specific cell types around the body.
- In situ gene therapy sees vectors directly infused into a target organ, such as the brain or liver.
- Ex vivo gene therapy sees vectors combined with a cell culture in the lab, before the modified cells are infused to the patient.
Step 3. Integration and Expression
Inside the cell, the vector must escape the endosome bubble to inject the genetic payload into the nucleus via a nuclear pore.
- Chromosomal integration sees the new genes fully incorporated into the host cell DNA. While this carries a risk of altering existing genes, it's more reliable for creating permanent gene expression.
- Episomal integration creates episomes, or short loops of DNA, which can attach to chromosomes during cell division to create long-lasting gene expression.
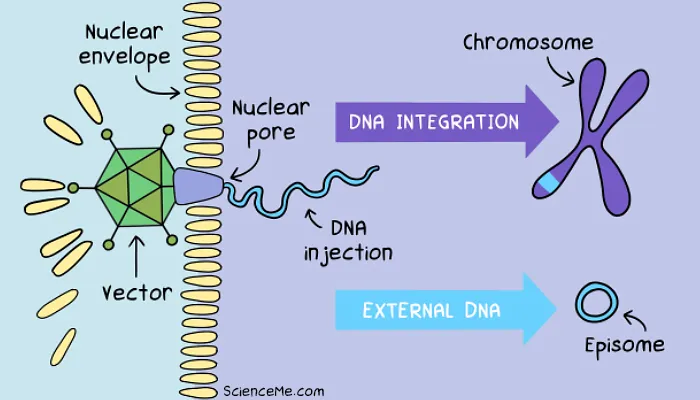
The vector escapes the endosome and injects the foreign DNA through a nuclear pore, where it either integrates with a host cell chromosome or persists separately as an episome.
Gene Therapy vs Genetic Vaccines
This may all be starting to sound like the same technology used in genetic vaccines. And you'd be right. So what's the difference between DNA and mRNA vaccines, like those developed for the SARS-CoV-2 virus, and your typical gene therapy?
Let's start with DNA vaccines (AstraZeneca, Johnson & Johnson). They're called DNA vaccines because they're based on the plasmid DNA (pDNA) of an adenovirus vector. To engineer the vaccine, the viral pDNA is modified with the removal of its own replication genes and the addition of antigen genes; in this case the spike genes of SARS-CoV-2.
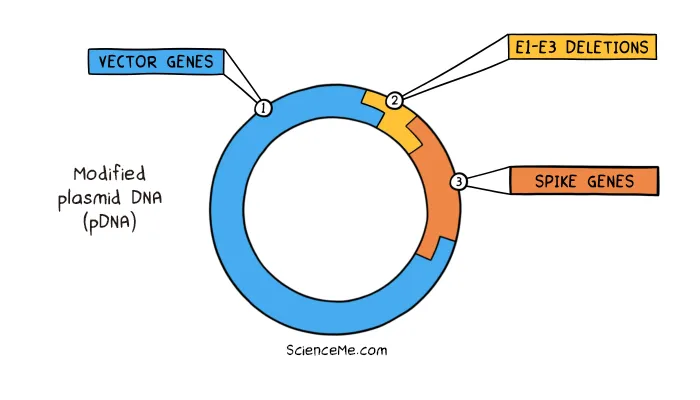
How to make a DNA vaccine: (1) splice open the vector plasmid DNA; (2) delete the E1-E3 regions needed for viral replication; (3) insert spike antigen genes.
Once injected into the muscle of your upper arm, the modified virus carries its payload into your muscle cells. The pDNA enters the nucleus, where the cell copies it into mRNA and expresses the viral spike proteins.
You'll be pleased to know that while pDNA is similar to episomal DNA, it lacks the native machinery to integrate or attach itself to chromosomes. When the cell dies, the pDNA is destroyed. This is why the genetic effect is short-term: the antigen is produced over several days or weeks, enough to train up the immune system.
The current mRNA vaccines (Pfizer-BioNtech and Moderna) use synthetic vectors called lipid nanoparticles (LNPs) to deliver genes to the cell cytoplasm. The cell translates the mRNA into viral antigens to train up your immunity. Note that mRNA doesn't enter the nucleus and is quicker to break down than pDNA.
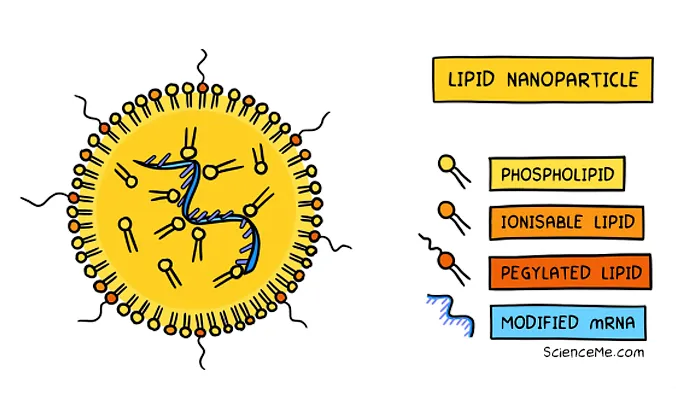
Current mRNA vaccines use lipid nanoparticles to deliver viral genes to the cell cytoplasm.
While genetic vaccines are based on the same principles and technology as gene therapy, they don't produce permanent gene expression.
So genetic vaccines have a short-term action by design. The same result would be a disaster in gene therapy, which must achieve permanent gene expression to be effective. Indeed, such long-term effects are incredibly difficult to achieve and the conservation of DNA remains one of the biggest challenges in gene therapy.
Gene Therapy | Genetic Vaccines | |
Genes | Human | Viral |
Vector | Viral/Synthetic | Viral/Synthetic |
Dosage | High (e.g. 3x1014 AAV) | Low (e.g. 5x1010 Ad) |
Delivery | Intravenous | Intramuscular |
Integration | Yes | No |
Expression | Permanent | Temporary |
The Challenges of Gene Therapy
The hard part of gene therapy is ensuring the new DNA is delivered to the cell nucleus intact, and then conserved through many cycles of cell replication. It's essential that the healthy genes are copied in dividing cells to produce the lasting therapeutic effects.
But we can't go in guns blazing. Scaling the delivery of gene therapy can induce a deadly immune response, while boosting gene expression can activate dormant cancer-causing DNA.
This is why most gene therapies are at the pre-clinical stage (ie, animal studies) or the clinical stage (ie, human trials), where they're examined for efficacy, side effects, adverse events, and long term outcomes.
There are many complex variables surrounding the technology itself. Depending on the disease, faulty genes may be swapped out entirely, repaired using selective reverse mutation, or deactivated through gene regulation. This necessitates different forms of genetic material, including DNA, mRNA, siRNA, miRNA, and others.
There's no one-size-fits-all solution to curing disease at the genetic level. This is why it will be many years before we have a full suite of gene therapy treatments.
The principles of gene therapy are elegant. Yet in practice, some elements of the execution have been described as crude—and as we'll see in a moment, vulnerable to catastrophic failure. Nonetheless, experts believe gene therapy will eventually become a staple of 21st century medicine.
The First Gene Therapy Trials
In 1986, Ashanti DeSilva was born without the ability to make a protein called ADA, which plays a crucial role in white blood cells.
At birth, Ashanti seemed like a healthy baby. But as she became exposed to bacteria and viruses in the environment, her ADA deficiency became manifest.
"Ashi had her first infection at just two days old. By the time she was walking, she was constantly hacking and dripping with coughs and colds." - Ricki Lewis, The Forever Fix: Gene Therapy and The Boy Who Saved It
Most infants with ADA deficiency don't survive past their second birthday. Ashanti was lucky. After being diagnosed with Severe Combined Immunodeficiency (SCID), she was put on protein replacement therapy using ADA sourced from cows.
Although the treatment was only partially effective, it kept Ashanti alive until she was four years old and recruited for one of the first gene therapy trials.
A sample of Ashanti's white blood cells was cultured in the lab and transfected with modified retroviruses carrying healthy ADA-coding genes. The sample was then infused into her bloodstream over the course of 20 minutes.
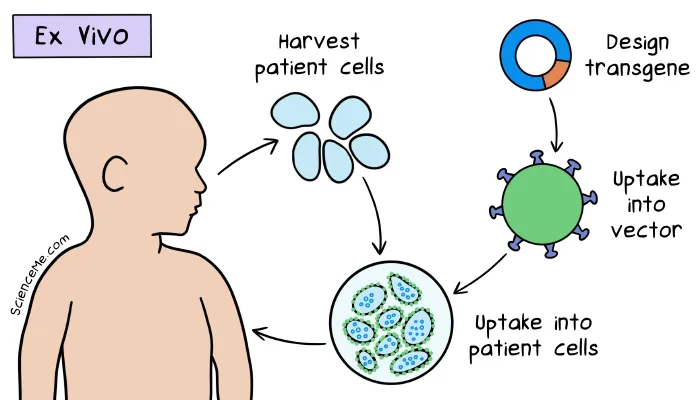
In Ashanti's ex vivo gene therapy, a retrovirus vector was modified with the deletion of viral replication genes and the addition of healthy ADA genes.
The gene therapy trial was a success. Millions of modified white blood cells began to produce ADA immediately, enabling Ashanti to make functional antibodies. The edited cells also went on to replicate with the new genes intact, providing long-term effects.
Although she needed 10 more infusions, Ashanti suffered no side effects from the ex vivo therapy, and was cured of SCID over the next two years. Today she's alive and well, married and with a Masters in Public Policy.
Ashanti's treatment prompted researchers to treat newborn babies with ADA deficiency in the same way. They even took white blood cells straight from the umbilical cord, enabling the infants to produce healthy immune cells from the start of their lives.
When Gene Therapy Goes Wrong
The first spectacular failure of gene therapy came nine years later, in 1999. It involved 18-year-old Jesse Gelsinger, who had a partial OTC deficiency which meant he couldn't effectively break down ammonia, a waste product of protein.
Without treatment, babies born with severe OTC deficiency die in the first few weeks of life. Jesse signed up to a gene therapy trial to help such infants, knowing he wouldn't be cured himself as the gene therapy effects would be transient.
The trial used the adenovirus 5 (Ad5) vector for delivery in situ, sending the virus directly to the liver for transfection. It had never been tested in humans before.
As the eighteenth and final patient in the trial, Jesse received the highest dose of the virus: 3.8x1013 (380 trillion) viral particles. It caused a violent immune response. Jesse suffered fever, blood clots, and widescale inflammation. Brain damage and organ failure ensued. Four days later, he died.
Some shocking revelations followed. The FDA investigation found that two other participants, who received lower doses than Jesse, had already suffered serious reactions to the adenovirus. This should have stopped the trial in its tracks.
Prior to the human trial, animal studies using a first generation vector saw two lab monkeys die from blood clots and liver inflammation. Jesse was never told this. When the family sued the trial organisers, they quickly settled out of court.
No matter how promising the technology, if gene therapy trials are poorly designed and executed, the results can be devastating.
Even with a good design, testing new drugs is inherently dangerous—that's why trials are necessary. Informed consent and proper regulation are critical parts of the process.
Although the tragedy led to tighter clinical trial regulations, Jesse was not the last to suffer from the experimental platform. Three years later, gene therapy hit the headlines again for the worst possible reasons.
The SCID Kids
Later trials would reveal the long term effects of gene therapy gone wrong.
"Most gene therapies are designed to achieve permanent or long-lasting effects in the human body, and this inherently increases the risk of delayed adverse events." - Gene Therapy Needs a Long Term Approach, Nature
In 2002, a gene therapy trial in France sought to treat babies suffering from X-SCID, another form of Ashanti's severe immunodeficiency disorder. They also received a retrovirus vector targeting complete chromosomal integration.
Initially, the infants responded well and the life-saving trial was deemed a success. But in the years that followed, 4 of the 9 children developed leukaemia, a cancer leading to the excessive production of abnormal white blood cells.
It was a baffling result. The FDA halted all gene therapy trials in the US until the cause could be identified.
Researchers realised the retrovirus vector had selectively inserted the new genes alongside cancer-causing genes.
Such genes start out as healthy DNA code used in cellular growth and repair. But if they mutate or express at high levels, they become cancerous oncogenes.
In the SCID trial, the therapeutic DNA included an enhancer region to boost expression. But the enhancer effect drifted thousands of bases downstream to activate dormant oncogenes as well.
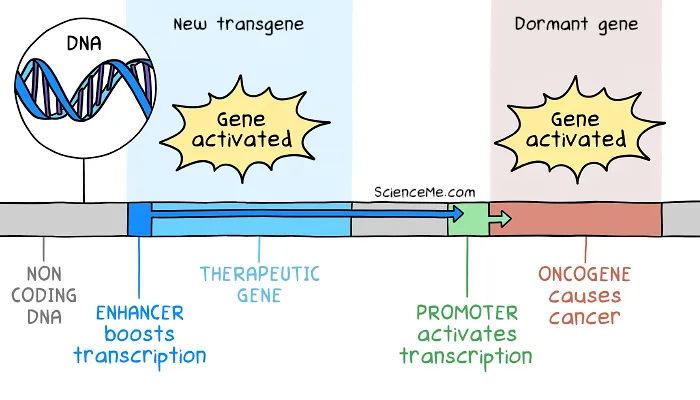
How gene therapy caused insertional mutagenesis in the SCID kids.
It's pretty damning when your experimental therapy starts giving children cancer. The episode was widely publicised in the media, and leukaemia eventually claimed the life of one of the children.
An independent investigation concluded that all integrating gene delivery models present the risk of such insertional mutagenesis.
It was a major blow to the field. The trial team raced to find a solution—not least because children were still dying of X-SCID, but the lead researcher himself was dying of cancer.
Their answer was to add an insulator to the therapeutic DNA. Genetic insulators serve as a barrier to limit the downstream effect of enhancers.
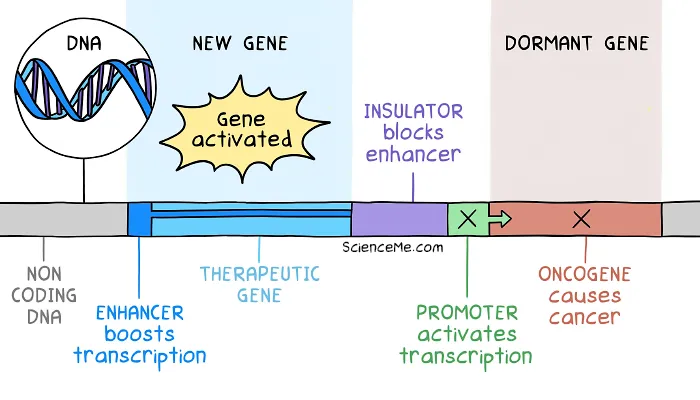
Genetic insulators prevent enhancer regions from activating downstream DNA and causing insertional mutagenesis.
Clinical trials resumed with the addition of this safety feature, and the evolution of gene therapy continued.
When Gene Therapy Saves Lives
Gene therapy saw major successes in the years that followed, saving the lives of numerous children born with rare inherited disorders. Here's one such example.
When Amy and Brad Price decided to have children, they had no idea they were both carriers of a single defective gene called ARSA. Their offspring would have a 1 in 4 chance of developing metachromatic leukodystrophy (MLD), a degenerative disease that destroys the brain's white matter, leading to paralysis, dementia, and death.
The first time they heard about MLD, Brad and Amy already had two children. One day, their three-year-old daughter, Liviana, complained that her legs didn't work.
Doctors soon diagnosed Liviana with MLD. But since she was already symptomatic, she was past the window for treatment. Testing of her baby brother, Giovanni, showed he had also inherited the disease.
This is common with MLD; families are only alerted to the faulty gene when an older sibling begins to show symptoms. While Liviana passed away from MLD, it provided a vital warning sign so her younger brother could be treated before the disease took hold.
In 2011, Italian doctors drew stem cells from Giovanni and transfected them ex vivo with a retrovirus vector carrying new ARSA genes. Five days later, the modified cells were returned to his body and started producing the missing enzyme.
Now a teenager, Giovanni has well surpassed the life expectancy for infantile MLD, and by all appearances is cured. Brad and Amy Price went on to have six more children, and when another daughter, Cecilia, was diagnosed with MLD, she also benefited from the life-saving gene therapy.
Cases like this provide hope to families in the face of rare childhood diseases. In recent years, gene therapy trials have successfully treated SCID, haemophilia, leukaemia, and blindness caused by retinitis pigmentosa (RP).
Is Gene Therapy Safe?
Successful gene therapy is heavily dependent on choosing the right viral vector, and delivering it at a safe but effective dose.
Take the widely-used adeno-associated virus (AAV) vectors. They were once celebrated for their ability to cross the blood-brain barrier, circumventing the need to drill into the skull to treat neurological disorders. But recent animal and human studies have thrown trusted AAV vectors into dispute.
In 2018, an AAV9 vector caused severe toxicity in primates and pigs, leading to liver and motor neuron damage.
The FDA halted human trials using high doses of AAV9 for muscular dystrophy (MD), and investigations into AAV toxicity are ongoing.
It's suspected that high-dose AAV gene therapy may cause DNA damage, stress to the endoplasmic reticulum (a cell organelle used in protein synthesis), and overexpression of transgenes in liver cells leading to liver failure.
To date, 149 gene therapies using AAV vectors have produced serious adverse events at a rate of 35%, with some resulting in death.
Another vector threat emerged in 2021. For the first time in gene therapy history, use of a lentivirus (a type of retrovirus) led to three children developing early-stage leukaemia.
Some suggest the lentivirus isn't to blame, but rather the unique addition of a promoter region triggering genes downstream. Lentiviruses have been used to treat more than 300 gene therapy patients for a dozen different conditions. If research can untangle the mechanism, lentiviruses may still be in the clear.
Discouraged by the risks of viral vectors, some researchers have turned to synthetic vectors like polymers, liposomes, and ultrasound-mediated microbubbles.
While these nanoscale carriers are currently less effective at delivering genes into cells, they are safer and cheaper, while presenting no DNA size limit.
But there's still a question of dosage. Consider the genetic mRNA vaccines that use small doses of lipid nanoparticles to deliver viral genes. This generates low level inflammation, which is ideal for kick-starting the immune system.
But gene therapy is about producing lasting genetic changes. This necessitates much higher doses of both vectors and genes. Scaling the delivery of synthetic vectors could also lead to life-threatening inflammation as seen in viruses.
The safety of gene therapy really depends on the genetic template, vector type, and dosage. Tweaking any variable in the wrong direction can lead to an excessive immune response, gene overexpression, or insertional mutagenesis.
The US now performs two-thirds of gene therapy trials in the world, and by 2025, the FDA is expected to approve 10-20 new gene therapies per year. Beyond rare childhood diseases, therapies are now targeted at common cancer, cardiovascular, infectious, and inflammatory diseases.
This changes the risk-benefit ratio considerably. Children with months to live may understandably be offered last-ditch experimental treatments. Yet the same may not apply to adults with chronic conditions and for which other treatments exist.
Gene therapy is a breakthrough proposition. But not all therapies pose equal risks. Until those risks are understood, each new trial poses a certain gamble in the search for a cure.
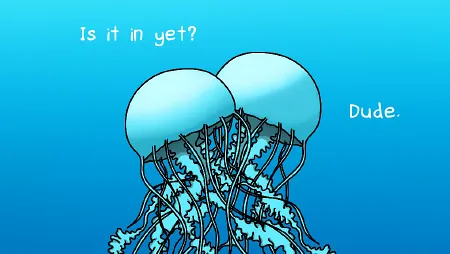
How Do Jellyfish Have Sex?
Jellyfish mastered sex long before us. Frankly, we're the ones odd-balling it with our penises, vaginas, and miserable childbirth.
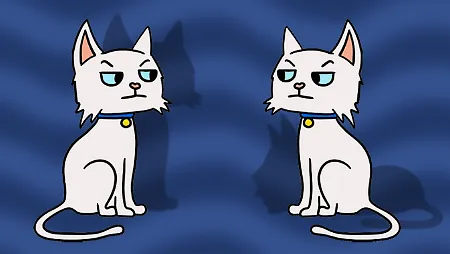
What is Schrodinger's Cat?
Schrodinger's Cat is a thought experiment created in 1935 by a brilliant scientist who loved quantum physics and hated cats.
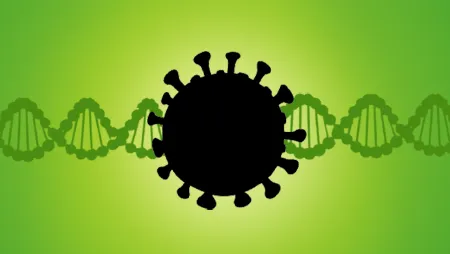
Viruses: Genes Gone Rogue
Viruses are runaway genes that meddle with our cells, also known as mobile genetic elements, intracellular parasites, or freeloading gits.
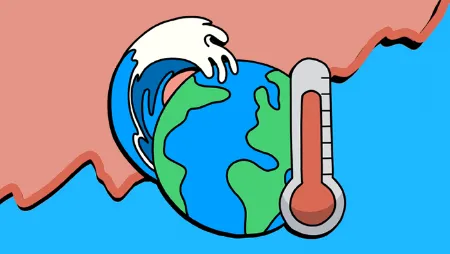
The State of Climate Change
An overview of natural and man-made climate change, including past, present, and forecast data on global temperatures and sea levels.
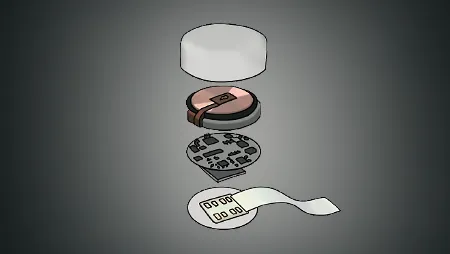
Neuralink and You
A Link is a coin-sized panel of microchips, a battery, and 3,000 electrodes along hair-thin neural threads. And it goes inside your skull.
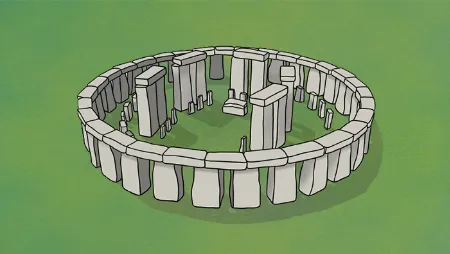
How Was Stonehenge Built?
In the 5,000 years since it was built, Stonehenge has been eroded by weather, sunken by earthworms, and picked at by handsy tourists.
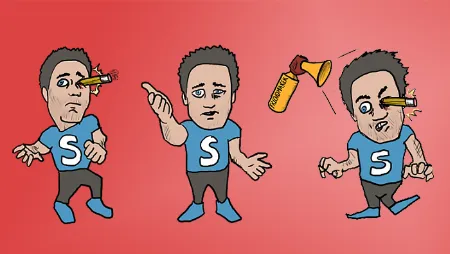
How Does Conditioning Work?
My friend Sutton here volunteered for a harmless experiment in classical conditioning. Great! Let's start by gouging him roughly in the eye.
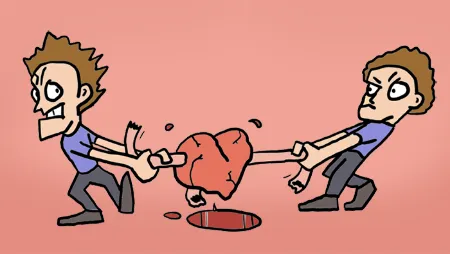
Who Owns Your Organs?
It's not you—it's me. Besides a pseudo-compassionate breakup line, it's also the answer to an ethical minefield of a question that arises when you die.
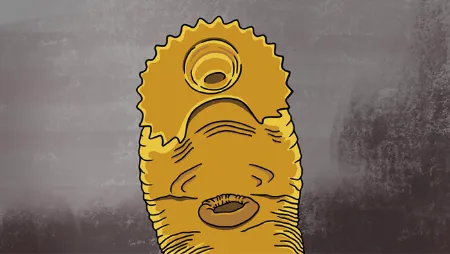
A Real Body-Snatching Parasite
Meet my associate, Curtuteria australis, a parasitic flatworm who takes three hosts during his convoluted lifecycle.
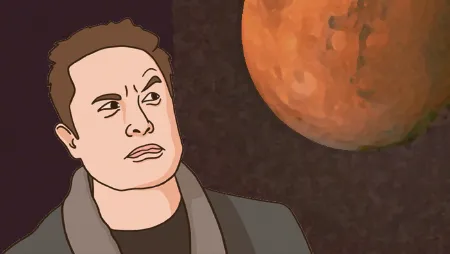
The Life of Elon Musk
So you have this dream of changing the world? For Elon Musk, this isn't enough. His ambitions are multiplanetary.